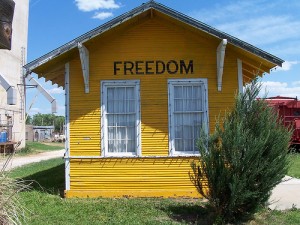
Freedom plays a central role in the practice of science. There is no one meaning to the word, “freedom,” that alone summarizes its role in science. We will begin with some basic definitions and then explore each meaning. Through such exploration will we arrive at a fuller understanding of how the freedom and science are interlocked.
Let us begin with a basic definition of science. There is much thought about the nature and practice of science, but in the modern understanding of what we call “the scientific method” there are three basic ingredients: “the recognition and formulation of a problem, the collection of data through observation and experiment, and the formulation and testing of hypotheses [1].” The scientific method is not a recipe; it’s a set of guidelines that allow for observers to develop a consistent approach to understanding the natural world. The method is predicated on the tenet that observation of the natural world, and formulation of theories that explain existing observations and predict new ones to be tested, is a consistent means to arrive at truth about the natural world. A “hypothesis” is a proposed explanation of a series of observations. A “theory” is a set of principles that result from hypothesis-testing, which itself creates new knowledge in that it explains observations rather than just reporting on them. After enough time has passed, and no violations of a theory have been observed, it is often raised to the level of a “law”.
While this sounds like an orderly progression, science is rarely orderly. The scientific method is not the pursuit of the lone investigator; rather, it is a social process with redundant self-correcting mechanisms. Individuals practicing the method may seek to advance their own views or biases; an essential component of science is the “review process,” where data, hypotheses, and theories are submitting to a community of peer experts for scrutiny. This happens in many layers: amongst one’s own local group of collaborators, with peer-reviewed journal editors and referees, and eventually with the whole communities of readers of the published work. Scientific practice by a lone investigator, or even a community of investigators, must still stand the long test of peer review. Even then, even decades or centuries later, old ideas may be found to be narrower than originally believed and absorbed into a larger theory which explains more observations and is based on a wider set of hypothesis testing. Science is a frontier ever in motion.
Within this framework, there are many freedoms which are central to the process. The ones which I will discuss here are: the freedom of the investigator to pursue their work, wherever it may lead; the freedom of others to criticize that work and demand more evidence; and the freedom to challenge authority in all of its forms, including the many tyrannies of popular belief, established scientific theory, and experts. This last one is a freedom often on display in the public square, especially surrounding issues that are uncomfortable to the general public: climate disruption, human sexuality and reproduction, and the evolution of biological life, to name a few. I’ll address this one last.
Let us begin with the freedom of the individual to pursue their own work. Central to the scientific process is the liberty of an investigator to apply the scientific method to a question of interest. There are many vague terms here, which I would like to define more clearly. By “liberty,” I mean the absence of restraint on the investigator to address their central question through the scientific method. By “interest,” I mean a question that bears on a subject of curiosity for the investigator, a subject of importance within a field of scientific inquiry, or a question that has obvious alignment with societal values.
Within this freedom, there is an interesting nexus that often leads to controversy: what happens when the freedom of the investigator meets the values of a society? There are two outcomes in that case: either the question under investigation is aligned with social values, and thus is accepted as a good; or, the question challenges existing social values, and is seen as controversial. There are many gradations in this scale. For example, the freedom to investigate a chemical means to disarm the “Lethal Factor” of Anthracis Bacillus would be seen as a social good. Anthrax is a potentially deadly biological weapon, and the lethal factor is the chemical secreted by the bacterium which ultimately leads to the fatal symptoms in humans. This is clearly aligned with a current societal good in the United States – the pursuit of countermeasures to biological terrorism. However, the development of a vaccine for the most prevalent forms of the Human Papilloma Virus (HPV), which causes the majority of cervical cancers in women, is also a social good (prevention of cancers) which has aspects that clash with other social values (HPV is sexually transmitted, and sex is a taboo in the U.S.). Human cloning, which may lead to the ability to regenerate fragile organ tissue, is widely viewed as primarily aligned against societal values in the U.S. There are societal concerns over questions of individuality and the ability to replace individuals by whole copy, and what that would mean for society, which have not been clearly addressed.
However, the freedom of the scientists to pursue the question to its conclusion is central to the kinds of innovation that have given the U.S. its current prosperity. Technological invention (computing, information, efficient manufacturing), biological invention (food science, for instance), and medical invention (new chemical and biological agents to treat disease), are all central in our economy. Not all questions have immediate application, but all questions are interesting to someone. The freedom to pursue those questions, however important or uncomfortable, is what enables discovery. It is discovery that is the driver of invention, and invention becomes the engine of economic growth and prosperity. If you do not have the freedom to question, if you are prevented from investigation, then there can be no discovery.
The second freedom is that of the scientific community to criticize or scrutinize the work of another scientist. Science requires both the freedom to inquire, and to be questioned by others. This is the “peer review” process mentioned above, and it is ultimately the most important self-correcting aspect of the scientific process. No claim can stand unchallenged, and scientists must be free to question all claims of discovery. There have been many false discoveries in the history of science: “the weight of the soul” claim by Dr. Duncan McDougal [2] and “cold nuclear fusion” [3] are a few well-known ones. It is the weight of peer review – both criticism of the original work and later efforts to independently reproduce the work – which leads to validation or invalidation of the original claim.
The reason this freedom is so important bears directly on the idea that the practice of science eventually leads to an understanding of “truth.” Truth is a prize held in the highest regard; it is the ultimate goal of science, of philosophy and religion, and of the legal system. Truth means something different to each of these endeavors; within science, a complete understanding of the natural world is the greatest outcome of investigation. If a discovery is claimed, then it must be questioned; for, if that is a false claim, then it does not help us to obtain the truth.
From a societal perspective, false claims and misdirection waste resources and lead scientists down a road that bends away from truth. Such information cannot lead to practical applications (because it is based on falsehoods) and thus stalls the engine of economic growth. Peer-review can’t prevent a bad idea from being aired, but it can prevent a bad idea from spawning worse ideas, or more importantly from leading investigators down alleys that will only ever be blind.
The freedom of one scientist to challenge the claims of another has served as the most important self-correcting mechanism of the scientific process; it doesn’t always work quickly, but it works. If we are not free to question each other’s evidence, hypotheses, and theories, then we cannot filter the truth from the mis-information.
The final freedom I wish to discuss here is the freedom to challenge authority. This is very much related to both of the previous freedoms discussed, but has some extreme and unique characteristics that make it important to discuss. Authority cannot be held in high-regard in the scientific community. Why? Because a pronouncement from authority does not, in and of itself, require the necessary ingredient of science: evidence. When a person, or even an idea, rises to the level of “authoritative,” then we risk assuming it will always be correct.
This assumption has proven wrong so many times, it’s impossible to conceive of a scientific process that has to respect authority. Witness the deference with which Newton’s Laws and the study of Mechanics (the orderly working of matter in a fixed framework of space and time) led most 19th-century physicists to assume that Maxwell’s Equations, which explained light, could not be complete. Maxwell’s Equations described a wave that traveled with the help of no medium; the laws of mechanics said all wave phenomena required a medium. It was physicists like Einstein who challenged the original assumption of the authority of Newton’s Laws that led to a better understanding of space and time, one which encompassed Newton’s Laws but defined a broader theory of nature.
This freedom is often mistaken in the public square are the freedom to arbitrarily challenge a scientist or scientific idea simply because we don’t agree with them. For instance, the theory of evolution – one of the strongest scientific theories in existence today – constantly is challenged in the public square even though within the scientific community there are no serious fundamental issues that have ever been identified with it. Certainly, there are many scientists who are actively testing the limits of the theory; but they do this by gathering data, forming hypotheses to explain the data, and seeing whether those hypotheses confirm the theory or challenge the theory. They are not challenging the theory simply because it challenges traditional societal values about the place of humans in the universe.
It is one thing to challenge scientific authority with evidence; it is quite another to challenge it with values or opinions. Values are sometimes built from evidence, but not always. Opinions, too, are sometimes evidence-based, but not always. The freedom to challenge authority is central to science – how else can we hope to come to a better understanding of the natural world if we are not free to challenge old ideas and assumptions? However, that freedom comes interlocked with a responsibility to do so only by the same means the authority was originally established: the scientific method.
There are many freedoms. The three I have described here are central to the scientific process. Scientists must be free to inquire, even if that inquiry challenges societal values. Scientists must be free to challenge each others’ claims. Without this, there can be no ascension of correct ideas and pruning of false ideas. Finally, there must be the freedom to challenge scientific authority, be it a scientist held in high regard or a scientific theory held in high regard. The responsibility that is locked to this freedom is that the challenge must apply the same tool: the scientific method. It is sweet poetry, that this central framework for developing new knowledge – the scientific method – both leads to the development of theories and laws and provides the means to challenge them in order to come to a better understanding of the truth. Freedom to inquire, to criticize, and to challenge is the glue that binds these things together.
[1] http://www.merriam-webster.com/dictionary/scientific%20method
[2] http://www.scribd.com/doc/20281719/21-Grams-Hypothesis-Concerning-Soul-Substance-Together-with-Experimental-Evidence-of-The-Existence-of-Such-Substance
[3] http://en.wikipedia.org/wiki/Cold_fusion
[4] Photo from http://www.flickr.com/photos/programwitch/2505184887/sizes/m/in/photostream/