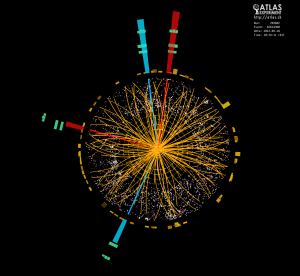
It was announced this morning (4:45am US Central time) that the 2013 Nobel Prize in Physics was given to Peter Higgs (University of Edinburgh, Scotland) and Francois Englert (Universite Libre de Bruxelles) for their “…discovery of a mechanism that contributes to our understanding of the origin of mass of subatomic particles…confirmed…by the ATLAS and CMS Experiments at CERN’s Large Hadron Collider.” I thought it might be useful to explain a little about the reason for this prize, and the tremendous experimental effort that confirmed the prediction that Higgs, Englert, and Robert Brout (now deceased), Gerald Guralnik, Carl Hagen, and Tom Kibble made almost 50 years ago.
The Fundamental Forces of Nature
To date, four fundamental forces of nature have been identified. These forces allow for interactions among the building blocks of nature, the “matter particles,” and thus for structure to emerge in our cosmos.
The most familiar force is gravity, but in the world of subatomic building blocks it plays the least important role and is the weakest of the four forces; we only know about gravity because we humans are big things, living on even bigger things (a planet), and we are made of so many subatomic building blocks that the force of gravity seems very important to us. But, to an atom, or something smaller than an atom, gravity is so weak as to have a seemingly meaningless impact on its day-to-day existence.
Also familiar to us is electromagnetism, which results in electric and magnetic phenomena in our day-to-day lives (lightning strikes, electricity, refrigerator magnets, lasers, telecommunications, etc.). The electromagnetic force is responsible for the stability of every atom in our body, holding the cloud of electrons in each atom in orbit around a compact central nucleus made from protons and neutrons. The electromagnetic force is transmitted by a particle, called the “photon,” which can travel forever in a straight line (if undisturbed) at the fastest speed we know – the speed of light. Photons have no mass; all of their energy derives from their motion.
Less familiar, but no less important than gravity and electromagnetism, are the strong and weak nuclear forces. The strong force, transmitted by particles called “gluons,” is very short-ranged and is responsible for binding protons and neutrons together inside the atomic nucleus. It’s also responsible for the fact that protons and neutrons are made from even more tightly bound fundamental building blocks, called “quarks,” which are the matter particles that actually respond to the very sticky gluons. Gluons are so sticky, they stick to themselves and cannot travel very far. While gluons are also without mass, they are confined to short distances around quarks and around themselves because they bind like crazy to those things.
The weak nuclear force is responsible for destabilizing the atomic nucleus, resulting in nuclear decay and radioactivity. Since the burning of the sun is, at heart, a series of nuclear processes (including nuclear decay), the sun would not work without the weak force. The weak force is also short in range, confined to distances about the size of an atomic nucleus (a millionth of a billionth of a meter). However, unlike the gluons, the “weak bosons” that transmit the weak force are not short-ranged because they are sticky; they actually just interact quite weakly, as their name implies. Rather, their short range is because they are very heavy, and cannot travel far as a result.
But herein lies the problem: the weak bosons shouldn’t have mass at all . . . at least, if you take quite literally the mathematical symmetries inherent in the quantum field theory that describes nature. According to those symmetries, all force carriers should have no mass – they should all be like the photon and the gluon. This is not what we see, however. The weak bosons have big masses, about 80-90 times heavier than the proton!
When you have a discrepancy like this between your explanatory framework (scientific theory) of nature and your observations of nature, this is not when you throw your hands up and quit! This is when you think very hard, very critically, and very creatively. And, always, you make testable predictions that can be disproven by an experimental test. This is precisely how this problem was solved, even if it required another five decades to finally and directly test the heart of the solution.
Broken symmetry and massive force particles
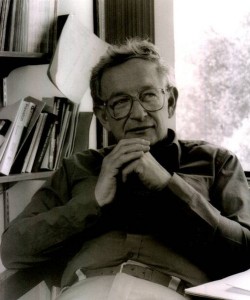
So it was that a series of papers in the 1960s [1] developed a proposal to solve this problem. The American physicist Philip W. Anderson recognized that a theory based on a field, whose constituents are massless force carriers, could develop behaviors consistent with massive force carriers if the field theory has a “degenerate vacuum” – that is, an infinite number of equally likely minimum energy states. Anderson was interested in understanding the behavior of plasmas, which are also described by a field theory -albeit one that is more classical than the one we now know describes the subatomic world. However, this idea could be applied to any field theory, something on which he commented at the end of his paper. Anderson later won the 1977 Nobel Prize in Physics for his work on the electronic structure of magnetic and disordered systems – that work led to the development of magnetic storage devices, like the computer hard drives that still pervade most inexpensive laptop and desktop computers. Anderson is a key example of how curiosity-driven research can change the world of the public by enabling innovative design and engineering built on novel, fundamental science.
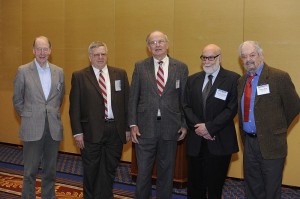
Applying this idea to a quantum field theory, like the one that describes the interactions of subatomic particles, was precisely what physicists Robert Brout, Francois Englert, Gerald Guralnik, Carl Hagen, Peter Higgs, and Tom Kibble did in a sequence of 1964 papers that followed on quickly after Anderson’s 1962 paper. The three independent groups (Guralnik, Hagen, Kibble; Englert and Brout; Higgs) developed the idea of broken symmetry (degenerate vacuum) in a quantum field theory and demonstrated that, indeed, force-carrying particles could obtain a mass. This idea solved the problem of the weak bosons and their short range. The Enlgert and Brout paper appeared first, in August of 1964; the Higgs paper appeared in October of 1964; the Guralnik, Hagen, and Kibble paper appeared in November of 1964. Their work was all conducted nearly simultaneously, however, and as they each published their papers they cited each other’s work in the order in which they appeared.
In this work, there was a bonus. Higgs recognized that a consequence of this mechanism was the appearance of another particle in nature. This is now typically called the “Higgs Boson,” since the mention of such a new and previously unobserved particle first appeared in Higgs’s 1964 paper.
Their work was not immediately accepted. There were competing ideas at the time and in subsequent years. It was decades before the Standard Model of Particle Physics, itself based on the basic ideas of quantum field theory and (broken) symmetries in nature, was established as experimental fact; it was decades longer before the Higgs Boson idea itself could even be directly tested.
The discovery of a new boson
The test of any theoretical idea is the grueling gauntlet of experimental science. Many a beautiful idea has been destroyed by the ugly fact of an experimental finding, to paraphrase the British biologist Thomas Henry Huxley. Yet, this interplay is the key to arriving at a reliable and consistent understanding of nature, and without it there is no meaningful and substantive progress in understanding the cosmos.
Experimental physicists have been searching for the Higgs Boson for a long time. It was originally assumed to be quite a light particle – perhaps weighing only a few times the mass of a proton. However, a dearth of evidence for such a particle meant that the community had to keep pushing the energy of their primary tools – particle accelerator and colliders – higher and higher. Doing so is no simple feat – it requires advancing simultaneously the frontiers of technology at all times, and in every direction – mechanical, electrical, mathematical, and computational. It requires the close collaboration of scientists and engineers.
In the 1980s and 1990s, a key machine in the search was LEP – the Large Electron-Positron Collider at CERN. The Tevatron at Fermilab was a key tool in the search for the Higgs Boson in the 1980s through the early 2000s. But without any strong evidence for the existence of a Higgs Boson within the reach of those machines, the Large Hadron Collider came online in 2010 with the express purpose of either finding this particle, or ruling it out up to the largest masses that are considered “mathematically tenable” for the Higgs Boson.
The size and scale of both the ATLAS and CMS collaborations is a testament to the global commitment to the fundamental science represented by particle physics, including the hunt for the Higgs Boson. ATLAS consists of 176 institutions spread across the globe, 44 of which are in the United States. CMS consists of 172 institutions spread equally across the globe, 48 of which are in the United States. Almost 3000 scientists, engineers, and students make up the membership of EACH of these two collaborations. Big questions . . . hard questions . . . whose answers have the potential to change our understanding of the universe are at the heart of all curiosity-driven science, and here in particle physics we have a large community centered on a global facility, built by many nations (including the U.S., who made significant contributions to the construction of the LHC and its experiments), served by the world community, and serving the world community in return. This is stunning, and its success has been enthralling – a testament to all large collaborations who daily face off against the toughest questions known to our species.
There has been a lot of excitement in the search for the Higgs. When the LEP Collider shut down in 2000, teams there thought they had some hints for the existence of the Higgs Boson at a mass of 115 times that of the proton. However, those hints weren’t convincing on their own, and required independent confirmation. The LHC experiments, ATLAS and CMS, presented tantalizing hints of a new particle with a mass between 120-130 times that of the proton in December of 2011, using just some of the data they would collect before the end of 2012. The Tevatron had already stopped taking data a few years earlier, but just a week before July 4, 2012, they held a large media event centered on twin seminars from the two competing experimental programs – CDF and D0 – showcasing their best results in the search for the Higgs Boson. They had tantalizing hints in the same mass range where the LHC experiments had shown intriguing results just half a year earlier, and using methods not yet accessible to the LHC experiments. But hints are hints, and in the realm of experimental science, one demands overwhelming evidence for extraordinary claims.
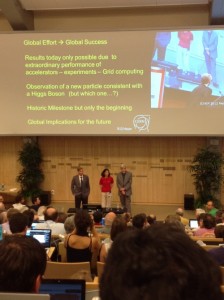
The definitive and overwhelming answer really came from the LHC data shown on July 4, 2012 (Higgsdependence Day, as some of us call it now). ATLAS and CMS showed evidence, beyond any reasonable doubt, for the existence of a new particle – a boson, like the photon or the weak bosons – with a mass of about 125 times that of the proton. When the announcements were made, first by Spokesperson Joseph Incandela of the CMS Experiment, then by Spokesperson Fabiola Gianotti of the ATLAS Experiment, the CERN Auditorium exploded in showers of applause and relief. Decades upon decades of searching for a particle consistent with the Higgs Boson hypothesis may have just drawn to a dramatic close, marking a new era of exploration and opportunity in our field. But was this newly discovered particle the Higgs Boson?
Quacks like a Higgs
The next year following the July 4, 2012 announcement was spent digging much more deeply into the nature of the new boson. More data, more tools, and more ideas were brought to bear on this question. If it’s a Higgs Boson, it should have no internal angular momentum . . . no “quantum spin.” If it’s a Higgs Boson, it should have a well-defined pattern of interactions with other bosons (like the weak bosons and the photon) and with matter particles (like the quarks). It took a tremendous international effort to discover the new particle, and no less of an effort to measure just some of its key properties.
What we have learned in the last year is that this new boson “quacks like a Higgs” [4] – its spin is most consistent with zero, and its pattern of interactions with matter and with other bosons matches very well to the Higgs hypothesis and poorly to competing hypotheses. In science, that’s evidence supporting the idea that this is a Higgs Boson, while refuting the claim that it’s something else – and that means we have a lot more confidence that this is, in fact, the Higgs Boson. In fact, the pile of evidence in favor of the Higgs Boson hypothesis is now so large it seems ludicrous that this particle could be anything else.
The Higgs Boson and the Future of Particle Physics
The particle physics community at the LHC is very focused now on continuing to measure the properties of the Higgs Boson with increasing precision. We’ve made tremendous strides, going a lot further already with less data at lower energy than originally planned for the LHC. But in 2015, the LHC comes back on at full strength (very close to, or at, its design energy) and at a blazing luminosity (a high, high rate of collisions). More energy means we are more likely, in each proton-proton collision, to make a Higgs Boson. More intensity means we crank up the rate at which protons collide. These two things together mean that the LHC will become the ultimate Higgs Factory for at least the next decade, if not longer. We will continue to study the Higgs and understand its properties. Perhaps the Higgs is even the gateway toward a more complete understanding of nature, one that at least includes a connection to the dark matter or the dark energy that pervade the cosmos but are undescribed (and unpredicted) by the Standard Model.
A key element of our work going forward will be to seek a uniting of the cosmic – dark matter and dark energy – with the subatomic. Such work can only be achieved by uniting the talents of particle physicists, astrophysicists, and cosmologists, to name just a few fields. We know that there are discoveries to be made beyond the Higgs, and we must pursue those discoveries, ever developing fruitful collaborations between these fields, ever pushing the bounds of technology, ever training new Ph.D. students who can go on to solve the hard problems facing our species, not just in physics but in all areas of study. The LHC is as much a factory for making new generations of clever, collaborative, and skillful problem-solvers as it is a factory for making Higgs Bosons.
The community is also looking beyond the LHC to find the best path forward toward a next-generation experimental program that continues to leverage the newly discovered Higgs Boson. We don’t quite know exactly what form that future will take, but one thing is certain. The 2013 Nobel Prize in Physics, awarded to some of the physicists who set this last revolution in motion, has set the stage for an even greater revolution in our understanding of nature. The Higgs Boson is like a new mountain, a great landmark that was predicted to exist and which now we see rising out of a new continent. We must explore that continent, and learn all of its secrets. But like all explorers, physicists also know this: now is the time to think past the Higgs, and to plan a path of exploration that leads from great Mount Higgs to the vast uncharted cosmic and subatomic lands that lie beyond.
[1] P. W. Anderson (1962). “Plasmons, Gauge Invariance, and Mass”. Physical Review 130 (1): 439–442.; F. Englert and R. Brout (1964). “Broken Symmetry and the Mass of Gauge Vector Mesons”. Physical Review Letters 13 (9): 321–323.; Peter W. Higgs (1964). “Broken Symmetries and the Masses of Gauge Bosons”. Physical Review Letters 13 (16): 508–509.; G. S. Guralnik, C. R. Hagen, and T. W. B. Kibble (1964). “Global Conservation Laws and Massless Particles”. Physical Review Letters 13 (20): 585–587.
[2] http://en.wikipedia.org/wiki/Philip_Warren_Anderson
[3] http://en.wikipedia.org/wiki/Higgs_mechanism
[4] Aad, G., et al. [ATLAS Collaboration]. “Observation of a new particle in the search for the Standard Model Higgs boson with the ATLAS detector at the LHC.” Phys. Lett. B 716 (2012) 1-29; S. Chatrchyan et al. [CMS Collaboration]. “Observation of a new boson at a mass of 125 GeV with the CMS experiment at the LHC.” Phys. Lett. B 716 (2012) 30-61; S. Chatrchyan et al. [CMS Collaboration]. “Observation of a new boson with mass near 125 GeV in pp collisions at $\sqrt{s}$ = 7 and 8 TeV.” J. High Energy Phys. 06 (2013) 081; Aad, G., et al. “Measurements of Higgs production and couplings using diboson final states with the ATLAS detector at the LHC.” Phys. Lett. B 726 (2013), pp. 88-119.; Aad, G., et al. [ATLAS Collaboration] “Evidence for the spin-0 nature of the Higgs boson using ATLAS data.” Phys. Lett. B 726 (2013), pp. 120-144.; S. Chatrchyan et al. [CMS Collaboration]. “Study of the mass and spin-parity of the Higgs boson candidate via its decays to Z boson pairs.” Phys. Rev. Lett. 110, 081803 (2013); CMS Collaboration. “Properties of the observed Higgs-like resonance decaying into two photons”. CMS-HIG-13-016.