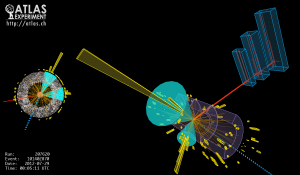
Today at the European Physical Society’s annual meeting, the ATLAS Experiment unveiled a number of new results based on the extensive data collected in 2015 and 2016 at a center-of-mass collision energy that is equivalent to balling together the energy of 13,000 proton masses. Among those results was one near and dear to my heart: the first evidence from the ATLAS Experiment of the Higgs decaying to its most favoritest decay mode, a pair of bottom quarks, also originally known as “beauty quarks.” But why did it take 7 years from the start of data collection to first see this decay, if the Higgs likes it so much? And why am I excited about it? Let’s find out!
The Amazing OctoHiggs
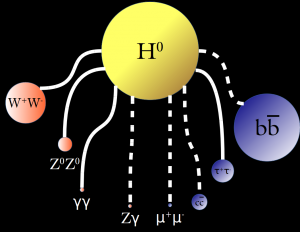
You can imagine the Higgs, and its most prominent and promising decay modes (the way in which its energy falls apart into other particles and the motion of those particles) using the “cephalopod diagram” I sketched up on the right. The Higgs decays to the things listed below it (there are more than these, but these are among the list of decays that we have the most hope of seeing at the LHC). The decay modes are represented by labeled circles. Red circles correspond to final states involving a “vector bosons” – one of the force-carrying particles of nature. These include W and Z bosons (carriers of the weak nuclear force) and the photon (carrier of the electromagnetic force). The blue circles represent fermion final states, those that result in matter building blocks: quarks and leptons. The area of the circle represents its relative proportion to the decay of the Higgs to bottom quark pairs; this is expected to be its largest decay mode, a whopping 60% of the decays of the Higgs!
While this decay — which we mathematically write using a “chemical reaction-like” diagram, — may be the most prominent one, it is also one of the most challenging. This is because most of what the LHC produces when it smashes protons together are quark and gluon “jets” – conical sprays of particles that result from the strong nuclear force and its effects on quarks and gluons when they are produced or liberated by the collider (see the graphic at the top of this post for a representation of such sprays). Every time the LHC brings two bunches of protons into collision, dozens of jets can result. The LHC smashes proton bunches together about 40 million times per second, roughly 10 months a year when it’s operating.
You can see the problem. Looking for a single Higgs boson, itself decaying to a pair of jets, out of all of those collisions is a painfully difficult task. I would liken it to looking for a pair of switchgrass blades in all the bales of hay in an industrial wheat field, which contains hundreds (or thousands) of bales. That’s about how hard this task was. That is why it took 7 years, from the first physics-quality proton-proton collisions until now, to even detect a glimpse of this process.
Make it, Break it
To watch a Higgs break apart into things like bottom quarks, one first has to make a Higgs. The LHC made about 2 million Higgs bosons in 2015 and 2016. However, most of those were produced solo, in a process known as “gluon fusion”. In fact, in the Higgs factory environment of the LHC, 87% of all Higgs bosons are made this way. This means that the Higgs would have been produced and then decay to a pair of bottom quark jets. But even in its own proton-proton collision, it would be surrounded by a dozen other jets from other processes occurring in parallel in each proton-proton collision. That one prized collision would itself be embedded in sea of billions upon billions of other proton-proton collisions, none of which contain a Higgs at all (but plenty of jets!). How does one attack this problem?
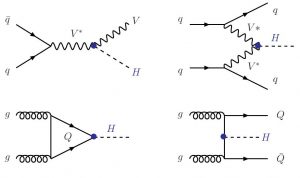
To make life easier, we try to select proton-proton collisions that are a bit rarer than those involving solo Higgs production. Such alternative production modes are like diamonds embedded in rock-salt — precious when you find them. Just 4% of the time a Higgs is produced, it is accompanied another special kind of particle – either a W or a Z boson. This is depicted in the figure to the right, in diagrams taken from Ref. [1]. The process we care about is in the upper-left of the four diagrams, and is known as “Higgsstrahlung” – the production first of a vector boson, V=W or Z, which then itself radiates an entire Higgs boson, much the way a high-velocity electron can radiate a photon (“Bremsstrahlung,” German for “braking radiation”). Bremsstrahlung is the basis of x-ray beams used in cancer treatment; Higgsstrahlung is the basis of fundamental discovery.
This Higgsstrahlung only occurs about 4% of the time, and since the production of W and Z bosons is already a much rarer process than many of the contaminating processes I mentioned above (e.g. events with nothing but quark and gluon jets in them), this greatly simplifies our challenge. It’s as if we know our two blades of switchgrass are going to be in a bale of hay held together with purple twine. Most of the bales will have standard brown twine, but if we find the bale with purple twine we can focus our search on just that bale, simplifying our task.
Even then, this is still a challenging search. There are still many more processes that yield W and Z bosons WITHOUT an accompanying Higgs, than there are Higgsstrahlung events. Dry switchgrass looks a lot like hay to the untrained eye! So we have to work really hard, using advanced statistical and classification techniques, such as boosted decision trees, to reject background processes and isolate promising signal-like proton-proton collisions (like that depicted at the top of this post).
(the numbers used in this section came from Ref. [2])
A glimpse of the favoritest decay
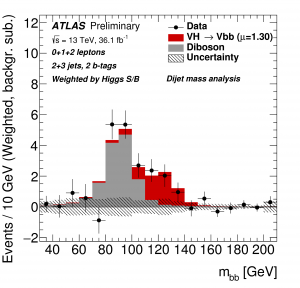
What we have seen from the 2015-2016 is considered just a “glimpse” of the prize, in a particle physics statistical sense. We denote our current level of certainly merely as “evidence” for the existence of this process. Numerically, that means that there is a 99.7% chance that this result is due to signal and not just due to background processes conspiring to fake the signal. You might be thinking, “Well, 99.7% certainty sounds pretty good!” It sounds good, but in statistical analysis this is considered only barely reliable as a result. To be really reliable – to really know that what we have seen is certainly Higgsstrahlung with bottom quarks in the final decay of the Higgs, we need to achieve 99.99994% certainty… which is only possible once we finish collecting the data we just began to get in June of this year. The newest round of data collection is ongoing and already looking really good – the LHC is doing an incredible job of delivering high-quality physics data for analysis, data that will great clarify this important process in nature. The LHC engineers and physicists, as well as the ATLAS engineers, technicians, and physicists, should be proud of what they have created and maintain, and what is has accomplished. Certainly, my gratitude goes to all of them.
But what good is it? Well, once you establish the favoritest decay mode of the Higgs, it becomes a lens – a big lens – through which we can view more carefully the Higgs boson. Not only that, but using this new big lens we can search for even rarer Higgs production processes than Higgsstrahlung, some of which may tell us about the Higgs quantum field itself. That field has a structure encoded in the standard model of particle physics, but how do we know that encoding is correct? This decay mode will help us answer that fundamental question.
Once firmly established beyond any doubt, the process of will become a powerful microscope that should let us peer deeper into the world of the Higgs boson. That excites me a great deal!
All physics is local
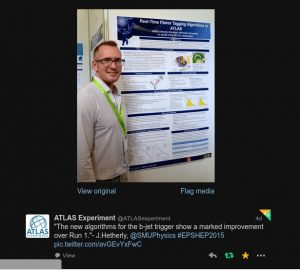
I am also excited about this result for other, more personal reasons. SMU has played a role in this glimpse. My second PhD student, Jeff Hetherly, completed his thesis in March on the first part of this search, using just the first third of the data set represented by this newest result. In that thesis, he helped lay the groundwork for other physicists to make this new measurement, especially as regards treatment of the shapes of background processes and how those are modeled in interpreting the data, and in the reduction of systematic errors due to the use of algorithms needed for identifying bottom-quark-initiated jets. In addition, SMU has a prominent role in the software development in the ATLAS Hbb group, and as a result of that role has supervised and coordinated the production of the data and simulation files used to achieve these results. Going forward, two SMU PhD students and one post-doctoral researcher will take on new roles in this analysis, and I expect we will be a part of the full 2015-2018 data analysis… and hopefully that means 99.99994% certainty (or greater) that this process exists in nature.
There is nothing more satisfying than a measurement, and I am proud we have been part of this (literally and figuratively) beautiful search, and I know that we will continue to be a part of it going forward.
UPDATE (July 7, 2017): The scientific paper documenting this analysis is now publicly available [4].
References
[1] A. Djouadi, “The Anatomy of electro-weak symmetry breaking. I: The Higgs boson in the standard model,” Phys. Rept. 457 (2008) 1–216, arXiv:hep-ph/0503172 [hep-ph].
[2] The LHC Higgs Cross Section Working Group Collaboration, D. de Florian et al., “Handbook of LHC Higgs Cross Sections: 4. Deciphering the Nature of the Higgs Sector,” arXiv:1610.07922 [hep-ph].
[3] https://atlas.cern/updates/physics-briefing/first-lhc-sighting-higgs-boson-its-favourite-decay
[4] https://atlas.web.cern.ch/Atlas/GROUPS/PHYSICS/CONFNOTES/ATLAS-CONF-2017-041/